Hydrogen-rich planetary materials
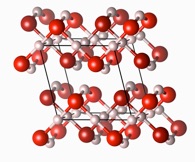
The phase diagram of water is extremely rich, with a large variety of crystalline phases found in experiment, depending on temperature and pressure conditions. As pressure exceeds 100GPa (or 1Mbar, one million atmospheres), water molecules are squeezed so tightly together that individual molecules become indiscernible. What happens if pressure is increased further? In our research we found a sequence of phase transitions that ultimately culminate in a metallic phase of ice, shown on the left. But not until pressures around 5TPa (or 50Mbar) are reached; this is more than ten times the pressure at the center of the Earth – but could be found in giant extrasolar icy planets.
See PNAS2012, PRB2013.
A more realistic picture of the interior of icy planets involves mixtures of small molecules, such as hydrogen, water, ammonia, and methane. At low pressures (up to 1GPa or 10kbar), these molecules form cage-like clathrate compounds. The clathrate hydrates of hydrogen and methane are of industrial interest, while others such as carbon dioxide hydrate could play an important role in environmental chemistry. We investigate the phase behaviour of such hydrates, often in collaboration with experimental colleagues, and employing innovative methods to accurately describe the weak interactions between the species.
See JCP2015, JPCL2017, JPCL2018.
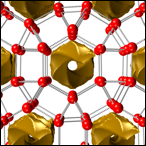
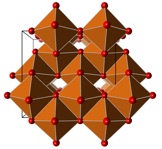
In Earth’s upper mantle pressures are somewhat higher (around 10GPa), and water can be stored in form of hydroxyl inside hydrous minerals. These minerals exhibit remarkable chemical variety, but understanding their phase behaviour at elevated pressures is crucial to model the water balance between mantle reservoirs and the surface. We apply crystal structure prediction to find high-pressure phases of hydrous minerals, which could not otherwise be identified.
See PNAS2016, PRB2016.
At even higher pressures (reaching 100s of GPa), more remarkable changes take place in molecular mixtures. In certain ammonia-water mixtures we predict a transformation from hydrogen-bonded molecular to fully ionic solids under pressure. This stabilises ammonia-rich hydrates up to pressures at the core boundary of Neptune, and should promote distinct layers within icy planets’ mantles. The efficient trapping of ammonia inside the planets’ interiors should influence atmospheric abundances.
See PNAS2017, JCP2018, Geo2019, JPCM2020, PRX2020.
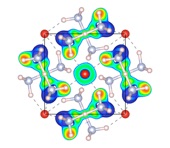
New materials at high pressures

The evolution of materials under conditions of high pressure and temperature is of fundamental interest in geo- and planetary sciences, but also a potential route towards synthesis of new useful compounds. Computational approaches now have the capability to predict with high accuracy possible stable (binary, ternary, etc) phases, independent of synthetic efforts, and evaluate their properties and eventual stability at atmospheric conditions. We led a comprehensive effort to sample the binary and ternary phase diagrams of combinations of light elements (lithium, beryllium, and boron), predicting new stable phases and explaining the properties of some of those already known.
See PRB2012, IC2012, PRB2012b, JACS2012, CEJ2013, JPCL2014, SRep2015.
Electronic excitations in solids and liquids
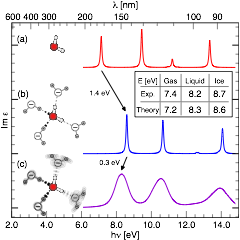
Contrary to most other materials, water, upon aggregation from the gas phase to the condensed state, increases its optical band gap. This sounds counter-intuitive, as one commonly finds a reduced band gap in the solid state due to the broadening of molecular energy levels into dispersive energy bands. In our research, we could show that this band dispersion is more than offset by an unusually strong Stark effect that shifts the center of the molecular energy bands. The inhomogeneous electric field causing this band shift is the sum of the dipole fields of the water molecules in the crystalline ice structure.
See PRL2008, PRL2011.
Relativistic effects in solids
For many materials, a non-relativistic description of their properties on an atomistic level (i.e., solving the Schrödinger equation), seems sufficient. However, as is well documented through the fine structure of atomic spectra, relativity does influence electronic energy levels, and hence also chemical and physical properties of the condensed state. Scalar-relativistic corrections (for the electronic kinetic energy and the Zitterbewegung) are responsible for valence s contractions, but also lead to valence d and f expansions. Spin-orbit coupling needs to be considered for heavy elements, as it roughly scales with Z2. We probe the effects of relativity on heavy elements’ solid state structures and their electronic properties, and disseminate the role the various relativistic contributions play. By predicting properties of condensed superheavy elements, we complement the single-atom chemistry experiments currently performed on these elements.
See PRB2010, PRL2013.
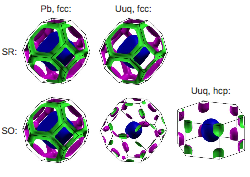
Weird quantum chemistry
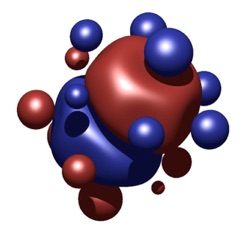
Finding the world’s highest coordinated atom: in “normal” molecules or clusters in the gas phase, the highest coordination number found for any atom is 12 – a regular icosahedral structure being exceptionally stable. For weakly interacting systems, higher coordination can be achieved, if the central atom is sufficiently “larger” than its ligands. We found (PbHe15)2+ to be very stable, with the shell of He atoms forming a distorted Frank-Kasper polyhedron (which, in turn, help to understand solid state structures of intermetallic compounds).
See ACIE2007.